QMC Quantum Microscopy: Advancing to the Heisenberg Limit
Technical Analysis | 06-06-2023 | By Liam Critchley
In the ever-evolving world of quantum technologies, researchers are constantly pushing the boundaries of what's possible. In a recent study titled "Quantum microscopy of cells at the Heisenberg limit," a team of scientists has made significant strides in the field of quantum imaging, presenting a new method known as Quantum Microscopy by Coincidence (QMC). This method promises to revolutionize bioimaging, offering super-resolution imaging at the Heisenberg limit with substantially higher speeds and contrast-to-noise ratios than existing wide-field quantum imaging methods.
As a science writer with a keen interest in chemistry, nanotechnology, and their intersecting disciplines, I, Liam Critchley, am thrilled to delve into the details of this groundbreaking research. With my background in Chemistry with Nanotechnology and Chemical Engineering, I'll be breaking down the complex concepts involved in this study and explaining how they could impact the future of bioimaging and cancer cell research.
Stay tuned as we explore the world of quantum microscopy, the unique properties of entangled biphotons, and the potential applications of these fascinating quantum phenomena in the realm of nondestructive bioimaging. Let's embark on this quantum journey together and discover how the smallest particles can make the biggest difference.
Emerging Applications of Quantum Technologies
Quantum technologies are starting to branch out into a number of application areas that go beyond quantum computing. Over the last few years, the integration of quantum mechanics and quantum principles into diagnostic technologies has become an area of intense research. There’s already been a number of studies performed on creating quantum sensors (where quantum wells are used to improve the sensing characteristics of the device), and another area that has been gathering interest is quantum microscopy.
Quantum microscopy uses entangled photons known as a biphoton state (the quantum entanglement of two photons so they become linked). The biphoton state has found a range of uses in other early quantum technologies, such as quantum computing, quantum metrology and quantum information applications.
When it comes to quantum microscopy, the entangled biphoton sources possess quantum (nonclassical) characteristics that can be used to improve a range of imaging techniques, including ghost imaging, quantum holography, and quantum optical coherence tomography. However, quantum imaging techniques that use a wide imaging field have so far had low speeds, poor spatial resolutions, and poor contrast-to-noise ratios.
The nonclassical nature of biphotons has motivated researchers to try and break classical limits. In studies so far, the diffraction pattern of biphotons has been shown to be half as narrow as classical light. This narrower diffraction pattern can be used in imaging approaches to achieve much higher resolutions than what is possible with classical light. This is why biphoton states are being investigated for different imaging methods, with a primary focus on medical applications.
According to a study published in Nature Communications[^1^], quantum technologies are starting to branch out into a number of application areas that go beyond quantum computing.
Biphotons in Quantum Imaging: Materials and Detectors
There have been a number of materials chosen to generate the biphoton states in quantum imaging applications. A number of nonlinear crystals have been used to generate the biphoton states, such as β-barium borate (BBO) and periodically poled potassium titanyl phosphate (PPKTP). There have also been a number of different detectors employed in quantum microscopes.
An example is single-photon avalanche diodes (SPADs) that provide direct coincidence measurements based on the time it takes for the entangled photon pairs to hit the detector. However, these are single-pixel detectors, so they don’t have spatial resolution. SPAD array cameras can be constructed to add spatial resolution to SPAD detectors, but they still only have a few pixels.
Other detectors that have gained use in quantum microscopy are electron-multiplying charge-coupled devices (EMCCDs), as they have a large number of pixels but cannot measure the coincidence of the biphoton directly. There are methods to determine the coincidence using the EMCCD detectors, but they require a lot of frames to produce a single coincidence image. Considering the infancy of the technology, and the low frame rate of EMCCDs, this can take a very long time (and more time than would ideally be desired for most research).
So far, the materials used in the entanglement generation and the detectors all have their own advantages and disadvantages. However, the field is still very new and new advancements are always being sought, with new studies coming out that advance the status quo.
One such study, titled 'Quantum microscopy of cells at the Heisenberg limit,' was conducted by a team of researchers led by Zhe He, Yide Zhang, and Xin Tong. Their work, published in Nature Communications, presents a new method known as Quantum Microscopy by Coincidence (QMC), which promises to revolutionize bioimaging by offering super-resolution imaging at the Heisenberg limit with substantially higher speeds and contrast-to-noise ratios than existing wide-field quantum imaging methods[^1^].
Figure 1: The Quantum Microscopy by Coincidence (QMC) Experimental Setup. This setup includes a continuous wave (CW), a Glan-Laser polarizer (GL), a half-wave plate (HWP), a variable wave plate (VWP), and a β-barium borate (BBO) crystal. It also features a 532 nm bandpass filter (BPF), a polarizing beam splitter (PBS), and an electron multiplying charge-coupled device (EMCCD) camera. The focal lengths are as follows: f0 = 50 mm, f1 = 180 mm, f2 = 9 mm, f3 = 300 mm, and f4 = 200 mm. The Fourier plane of the BBO crystal, denoted as P0, is set as the source Fourier plane. This intricate setup is the heart of the QMC method, enabling the groundbreaking imaging capabilities we're about to delve into.
Pushing the Boundaries: Quantum Microscopy Beyond the Classical Limit
The quantum entanglement of photons can be used to achieve a resolution beyond the classical limit. When this happens, x number of photons entangled can improve the spatial resolution x number of times at the Heisenberg limit—the optimal rate where the accuracy of the measurement can scale with the energy used in the measurement. The Heisenberg limit is the true quantum limit and cannot technically be surpassed, but accuracy levels and resolutions can be up to the Heisenberg limit. Some studies have also proffered that the Heisenberg limit could be reached in quantum microscopes with the need for both entangled photons to pass through the object being imaged.
Researchers have now developed a wide-field imaging quantum microscopy approach, known as quantum microscopy by coincidence (QMC), using EMCCD cameras as the detector. The research has made some advancements in previous studies, as many other efforts could not be used in practical microscopy applications due to not offering high-resolution imaging or through having a low imaging speed that requires a large number of frames to perform the coincidence measurement.
Figure 2 provides an insight into the coincidence measurement in Quantum Microscopy by Coincidence (QMC). Part (a) explains that this measurement hinges on the larger covariance between entangled photons across a series of frames, compared to that between two random photons. The left and right regions of the EMCCD, denoted as L and R, are used for detecting the signal and idler photons. The symmetric positions on the detector for these photons are represented as r2,s and r2,i, while r′2,i marks a random position in the right region, distinct from r2,i. The inset shows the intensities (I) at these three positions in various frames. In part (b), the contrast-to-noise ratios (CNRs) of QMC and the wide-field quantum imaging methods from referenced studies are compared using different frame counts. The data is presented as means with standard errors of the means (n = 10). Part (c) further contrasts the CNRs of QMC and the wide-field quantum imaging methods with 105 frames under the influence of stray light of varying intensities. The data is again presented as means with standard errors of the means (n = 10). The average number of photons incident on the EMCCD is 0.49 per pixel per frame. Finally, classical (d) and QMC (e) images of carbon fibres, taken in the presence of stray light with an intensity of 8I0 and using 2 × 106 frames, are presented. The scale bars represent 20 μm.
In this study, researchers have built on previous efforts to deliver a more practical quantum microscopy system that has a better spatial resolution and speed by using a higher numerical aperture and a more efficient algorithm. The QMC system relies on the nonclassical properties of the biphoton entanglement to provide balanced pathways, a super high resolution (1.4 μm) at the Heisenberg limit, speeds that are five times greater, a contrast-to-noise ratio that is around two and a half times higher, and a resistance to stray light that is ten times greater than existing wide field quantum imaging techniques.
According to the study, 'QMC benefits from a configuration with balanced pathlengths, where a pair of entangled photons traversing symmetric paths with balanced optical pathlengths in two arms behave like a single photon with half the wavelength, leading to a two-fold resolution improvement.' This finding is particularly significant as it demonstrates the potential of QMC to revolutionise bioimaging.
Drawing from my experience in the field of nanotechnology and chemical engineering, I find the approach taken by the researchers in this study to be particularly innovative. The use of EMCCD cameras as detectors in the QMC system represents a significant advancement in the field. This could potentially open up new avenues for research and application in the realm of quantum microscopy.
Compared to other quantum imaging techniques that operate at the standard quantum limit (which is different to the Heisenberg limit where the precision at the Heisenberg limit is much better and absolute), the resolution is improved two-fold because the balanced pathways in this QMC method traverse symmetric pathways with balanced optical pathlengths and behave like a single photon with half the wavelength—leading to a resolution improvement because smaller wavelengths provide less interference and can resolve finer details than longer wavelengths of light.
In terms of practical applications, the researchers focused their efforts towards non-destructive bioimaging applications. The researchers used the quantum microscope to image cancer cells using a low-intensity illumination with a 1.4 μm resolution and a 100 × 50 μm2 field of view. This imaging approach revealed fine features in the image that cannot be resolved by classical microscopy methods. These advancements in quantum microscopy push beyond what is possible with both classical systems and other quantum microscopy methods by pushing the accuracy to the Heisenberg limit, and if it can be done in one quantum microscopy system, then it is possible that it can be transferred to other quantum microscopes as well as for pushing the boundaries of classical high-resolution imaging systems by introducing quantum enhancements.
The implications of this research are vast. The ability to image cells at the Heisenberg limit could revolutionise our understanding of cellular structures and processes, potentially leading to breakthroughs in disease diagnosis and treatment. Furthermore, the nondestructive nature of QMC makes it particularly promising for cancer cell research, as it allows for the detailed study of cancer cells without damaging them. Looking forward, the researchers behind the study are optimistic about the potential of QMC to further advance the field of quantum microscopy[^1^].
In conclusion
The field of quantum microscopy is on the cusp of a major breakthrough, thanks to the development of Quantum Microscopy by Coincidence (QMC). This new method, which offers super-resolution imaging at the Heisenberg limit, has the potential to revolutionise bioimaging and cancer cell research. As we continue to push the boundaries of quantum technologies, it's exciting to imagine what other breakthroughs lie ahead.
Reference:
- He, Z., Zhang, Y., Tong, X., Li, L., & Wang, L. V. (2023). Quantum microscopy of cells at the Heisenberg limit. Nature Communications, 14(1), 2441. https://doi.org/10.1038/s41467-023-38191-4. [^1^]
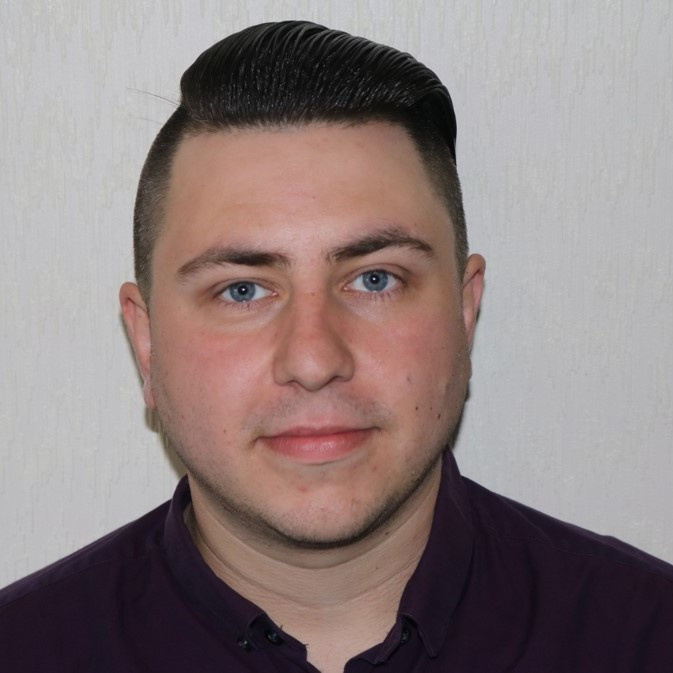