GaN's Barrier to Semiconductor Supremacy: The P-Type Problem
Insights | 06-02-2024 | By Robin Mitchell
A close-up photograph capturing a silicon wafer in the midst of production at an advanced semiconductor foundry specialising in microchip manufacturing.
Key things to know:
- Gallium Nitride (GaN) is emerging as a leading semiconductor material for power and RF applications due to its superior efficiency and performance over silicon.
- The absence of P-type GaN devices presents a significant challenge, limiting the material's potential in various electronic components and systems.
- GaN's high electron mobility and large bandgap enable it to operate at higher frequencies and voltages, making it crucial for RF power applications.
- Advancements in GaN technology could revolutionise energy-efficient electronics, but the lack of P-type devices remains a critical hurdle to overcome.
As the world looks for new opportunities in the realm of semiconductors, Gallium Nitride continues to stand out as a potential candidate for future power and RF applications. However, for all the benefits that it provides, it still faces a major challenge; no P-type offerings. Why is GaN touted as being the next major semiconductor material, why is the lack of P-type GaN devices a major drawback, and what does this mean for designs in the future?
Understanding the implications of the absence of P-type GaN devices requires a deeper dive into semiconductor physics and the role of P-N junctions in electronic devices. The synergy between P-type and N-type semiconductors forms the foundation of most electronic components, from diodes to transistors, enabling a wide range of functionalities including switching, amplification, and modulation. The lack of P-type GaN limits the design and efficiency of such components, necessitating alternative or hybrid solutions that may not fully leverage GaN's inherent advantages.
Why is GaN touted as being the next major semiconductor material?
In the field of electronics, four facts have remained true ever since the first electronic devices went onto the market; they need to be made as small as possible, as cheap as possible, provide as much power as possible, and consume as little power as possible. Considering that these requirements are often at odds with each other, trying to create the perfect electronic device that can satisfy these four requirements is something of a pipe dream, but that hasn’t stopped engineers from making every effort to achieve it.
Using the four guiding principles, engineers have managed to achieve all kinds of seemingly impossible tasks, with computers being shrunk down from room-sizes devices into chips smaller than a grain of rice, smartphones that allow for wireless communication and access to the internet, and virtual reality systems that can now be worn and used independently from a host machine. However, as engineers approach the physical limits of commonly used materials (such as silicon), making devices smaller and using less power is now becoming an impossibility.
As such, researchers are constantly looking for new materials that may be able to replace such common materials and continue to provide smaller devices that operate more efficiently. One such material that has caught the eye of many is Gallium Nitride (GaN), and when compared to silicon, it’s pretty obvious why. For a deeper understanding of how GaN fits into the broader landscape of power electronics, explore our detailed overview here.
The Superior Efficiency of Gallium Nitride
To start, GaN is able to conduct up to 1000 times more efficiently than silicon, allowing it to work at higher currents. This means that GaN devices are able to operate at significantly higher powers without generating as much heat, thereby allowing them to be made smaller for the same given power.
The thermal management advantages of GaN, despite its slightly inferior thermal conductivity compared to silicon, open new avenues for high-power electronics. This is particularly relevant in applications where space is at a premium and cooling solutions need to be minimized, such as in aerospace and automotive electronics. The ability of GaN devices to maintain performance at elevated temperatures further underscores their potential in harsh environment applications.
Secondly, the larger bandgap of GaN (3.4eV compared to 1.1eV) allows for use at higher voltages before experiencing a dielectric breakdown. Thus, not only is GaN able to provide greater powers, but do so at higher voltages while maintaining a far greater efficiency.
The high electron mobility also allows for GaN to be used at far greater frequencies. This factor makes GaN crucial for RF power applications that operate well above the GHz range (something that Si can struggle with).
However, when it comes to thermal conductivity, silicon is marginally better than GaN, meaning that GaN devices have somewhat larger thermal requirements compared to silicon devices. As such, this lack of thermal conductivity imposes a limit on the ability to shrink GaN devices when operating at high power (as bulk material is needed to dissipate the heat).
GaN’s Achilles Heel – No P-Types
Having a semiconductor capable of high-power operation at high frequency is all fine and good, but for all the benefits that GaN provides, there is one major drawback that has seriously hindered its ability to replace silicon in numerous applications: the lack of a P-type.
Arguably, one of the prime purposes of these newly discovered materials is to provide major efficiency boosts as well as supporting higher powers and voltages, and there is no doubt that current GaN transistors can provide this. However, while a single GaN transistor is certainly capable of providing some impressive features, the fact that all current commercial GaN devices are N-type impacts their ability to be extremely efficient.
To understand why this is the case, we need to look at how NMOS and CMOS logic work. NMOS logic was a highly popular technology throughout the 1970s and 1980s due to its simplistic manufacturing process and design. By using a single resistor connected between the power and drain of an N-type MOS transistor, the gate to that transistor is able to control the voltage at the drain of the MOS transistor, effectively realising a NOT gate. If combined with other NMOS transistors, it becomes possible to create all logic elements, including AND, OR, XOR, and latches.
However, while this technology is simple, the fact that it uses a resistor to provide power means that when an NMOS transistor conducts, large amounts of power is wasted across the resistor. For a single gate, this power loss is minimal, but when scaled across a small 8-bit CPU, this power loss can add up, making the device hot and limiting the number of active devices on a single chip.
The Evolution from NMOS to CMOS Technology
CMOS, on the other hand, utilises a P and N type transistor that work together in opposing ways. Regardless of the input state of a CMOS logic gate, the output of the gate will never allow a connection from power to ground, significantly reducing power losses (as when the N-type conducts, the P-type is insulative, and vice versa). In fact, the only real power loss in a CMOS circuit is during state switches, where a momentary connection between power and ground is formed through the complementary pair.
Going back to GaN devices, as only N-types currently exist, the only technology currently available to GaN is NMOS, which itself is extremely power hungry. For an RF amplifier, this isn’t an issue, but when it comes to logic circuits, it is a major drawback.
The quest for energy efficiency in electronics has never been more critical, with global energy consumption on the rise and the environmental impact of technology under scrutiny. The limitations of NMOS technology in terms of power consumption highlight the urgent need for breakthroughs in semiconductor materials that can offer both high performance and energy efficiency. The development of P-type GaN or alternative complementary technologies could mark a significant milestone in this quest, potentially revolutionising the design of energy-efficient electronic devices.
Interestingly, it is perfectly possible to create a P-type GaN device, and these have been used in blue LED sources, including Blue Ray, but while these are adequate for optical electronics, they are far from ideal for use in digital logic and power systems. For example, the only practical dopant for creating P-type GaN devices is magnesium, but as high concentrations are required, hydrogen can easily ingress into the structure during annealing, which affects the performance of the material.
Thus, for the time being, as P-type GaN devices are not available, engineers are unable to utilise the full potential of GaN as a semiconductor.
What does this mean for engineers in the future?
Currently, there are numerous materials being looked at, with another major candidate being Silicon Carbide. Like GaN, it offers higher voltage operation, higher breakdown, and better conductivity compared to silicon. Furthermore, its high thermal conductivity allows for it to be used in extreme temperatures as well as being made significantly smaller while simultaneously controlling far greater powers.
But unlike GaN, SiC is not suitable for high frequencies, meaning that it will unlikely be used in RF applications. Thus, GaN remains the top choice for engineers looking to create small power amplifiers. One solution to the P-type issue is to combine GaN with P-type silicon MOS transistors, and while this does provide complementary capabilities, it inherently limits the GaN in terms of frequency and efficiency.
As technology progresses, it is likely that researchers will eventually find either a P-type GaN device or a complementary device using a different technology that can be combined with GaN. However, until that day arrives, GaN will continue to be limited by the technology of our time.
The interdisciplinary nature of semiconductor research, involving materials science, electrical engineering, and physics, underscores the collaborative efforts required to overcome the current limitations of GaN technology. The potential breakthrough in developing P-type GaN or finding a suitable complementary material could not only enhance the capabilities of GaN-based devices but also contribute to the broader field of semiconductor technology, paving the way for more efficient, compact, and reliable electronic systems in the future.
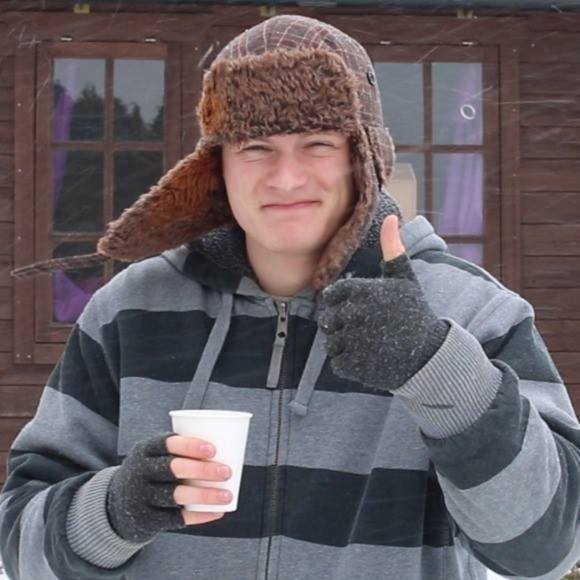