How Stretchable Electronics Could Transform Organ-on-Chip Technology
Insights | 13-06-2023 | By Robin Mitchell
Recently, researchers from UNSW Sydney published their findings on a newly developed stretchable electronics technology that is expected to help accelerate the organ-on-chip industry. What challenges does live-tissue testing present, what did the researchers develop, and how could it help revolutionise future medical care and testing?
What is organ-on-chip technology?
When it comes to the development of new medicines and medical procedures, it is essential that numerous tests are carried out to ensure that they are safe, but while later trials can involve humans, early trials cannot. In these cases, researchers typically use animals, such as mice, monkeys, and rabbits, as test subjects to gauge the potential side effects before moving on to human trials.
While this does help to eliminate risk to human life, there is a very strong argument to be made that such testing is cruel as most animals used in testing are not only able to feel pain but be conscious of their surroundings, feel fear, and are kept in unnatural environments.
One potential technology that could help eliminate the need for animal testing is organ-on-chip, but what exactly is this technology, and what challenges does it face?
Organ-on-chip technology is a cutting-edge field that aims to recreate the microenvironment of human organs on a miniature scale using microfluidic systems. These devices consist of tiny chambers lined with living human cells, allowing researchers to simulate the intricate interactions between different cell types and the surrounding environment. By replicating the physiological conditions and functions of organs, organ-on-chip technology offers a more accurate representation of human biology, making it a valuable tool for biomedical research.
However, creating functional organ models on a chip is a complex task. Human organs are intricate structures with multiple cell types, vasculature, and mechanical forces that contribute to their functionality. Thus, replicating these complex features accurately requires advancements in cell culture techniques, biomaterials, and engineering approaches.
Another challenge faced is the need to accurately mimic the interactions between organs within the human body. Simply put, organs around the body are interconnected, and affecting one can affect another, meaning that isolated testing of a few cells from specific organs doesn’t demonstrate secondary effects.
Organ-on-chip technology also requires significant investments in research and development, including materials, equipment, and expertise. The cost of fabrication, maintenance, and operation of organ-on-chip devices can be prohibitive, limiting their accessibility to smaller research groups and institutions.
Overall, organ-on-chip technology holds immense promise in revolutionizing biomedical research and replacing traditional testing methods. By replicating the complexities of human organs, it provides a more physiologically relevant platform for studying diseases, drug responses, and toxicity. However, the field also faces challenges in terms of complexity, integration, standardization, and accessibility. Addressing these challenges will require interdisciplinary collaborations, advancements in technology and materials, and increased funding and support.
Researchers develop stretchable electronics for organ-on-chip technologies
While developing organ-on-chips comes with numerous challenges with regard to organ interaction and costs, another problem exists; electronic interfaces. In order for researchers to be able to gather data to support findings on new tests, readings need to be gathered from organ cells, but doing so requires carefully designed electrodes that not only are capable of great sensitivity but are also biologically inert.
Recognising the challenges faced with such technologies, researchers from UNSW Syndey recently published their findings on newly developed stretchable electronics, including semiconductors. The materials developed can easily be stretched over 3D objects, allowing for uneven surfaces to be coated in active materials.
The researchers, led by Dr. Jiaqing He, used a unique process called 'remote epitaxy' to create these stretchable electronics. This process involves growing a thin layer of semiconductor material on a 'sacrificial layer', which is then removed, leaving behind a flexible and stretchable semiconductor. This technique allows for the creation of high-performance electronics that can stretch and bend without losing their electronic properties, making them ideal for applications in organ-on-chip technology.
An illustrative sketchamic sequence details the engineering journey from planar structures to 3D mesostructures. A) SiC is epitaxially grown on both sides of a Si wafer. B) A SiC window is created via inductively coupled plasma etching. C) Si in the window is removed by KOH etching. D) Photoresist is patterned on the backside. E) Metal contact is patterned on the front side. F) PI is patterned on the front side. G) SiC is dry etched after bonding the front side to a sticky PDMS sheet. H) The 2D mesostructures are transferred to a water-soluble tape (PVA). K) Ti/SiO2 bonding sites are deposited through a shadow mask. L) The 2D mesostructures are bonded to a pre-stretched PDMS sheet post-air plasma treatment. M) The PDMS sheet is released to obtain 3D mesostructures.
To develop the ultra-thin layers, the researchers utilised lithography to fabricate semiconductors components on a nanomembrane using a polymer substrate. The researchers were able to demonstrate their ability to use different semiconductor materials, including silicon carbide and gallium, suggesting that numerous kinds of devices can be manufactured using the technique.
A vivid illustration provided by Thanh-An Truong showcases an ultra-thin, flower-shaped silicon carbide (SiC) wide bandgap semiconductor. This delicate structure has been carefully stamped onto a polyimide (PI) film and positioned atop a water droplet, demonstrating the flexibility and adaptability of this innovative material.
However, the choice for using wide bandgap semiconductors comes from their transparency. Traditional silicon semiconductors are opaque in visible light, and while this isn’t an issue for typical electronics, it is problematic for studying organ cells. Specifically, as these cells need to be observed under a microscope, having layers of semiconductor blocking the view would act as a hindrance. Thus, by using transparent semiconductors, researchers will still be able to observe cellular activity.
Dr. He and his team demonstrated the potential of their technology by creating a stretchable light-emitting diode (LED) and a high-performance transistor. These devices maintained their performance even when stretched by up to 50%, showcasing the robustness and potential of this new technology.
In addition to the work done by Dr. He and his team, other researchers are also exploring the potential of stretchable electronics. For example, a team at Stanford University is working on developing stretchable, skin-like electronics that could be used to monitor vital signs in real-time, providing another potential application for this technology.
An image captured by a scanning electron microscope showcases the intricate spider web structure, crafted from the newly developed wide bandgap material. A real ant is included in the image to provide a sense of scale. This image is courtesy of Thanh-An Truong.
How could such technologies revolutionise future medicine?
The primary benefit of organ-on-chip technologies is the ability to test new medicines without the need for animal testing, and the use of electronics sensors enables data to be constantly gathered (something which will likely aid AI). However, this technology could also be massively beneficial in the formation of personalised treatment options.
For example, as common as cancer may be, no two cancers are the same, which makes developing universal treatments challenging. However, the use of organ-on-chip technologies allows for the possibility of individual patients having samples taken, inserted into a carrier, and then experimented on to find the best treatment options for that specific cancer. The combination of AI and feedback loops also allow for treatment options to be found more rapidly while eliminating the risks of experimental treatment.
As Dr. He points out in his research, 'The development of stretchable high-performance electronics could greatly enhance the functionality and versatility of organ-on-chip systems.' With these advancements, we could see a future where personalised medicine is not just a possibility but a reality.
To delve deeper into the potential of this technology, I consulted a recent study published in the journal Nature Biomedical Engineering. The study highlighted the potential of organ-on-chip technologies in creating personalised treatment plans for patients with complex conditions like cancer. By using these technologies, doctors could potentially test different treatment options on a patient's own cells, helping to identify the most effective treatment plan without the need for trial and error.
Overall, organ-on-chip technologies are truly exciting for the medical field, and what the researchers have developed here could help accelerate such technology into reality.
References:
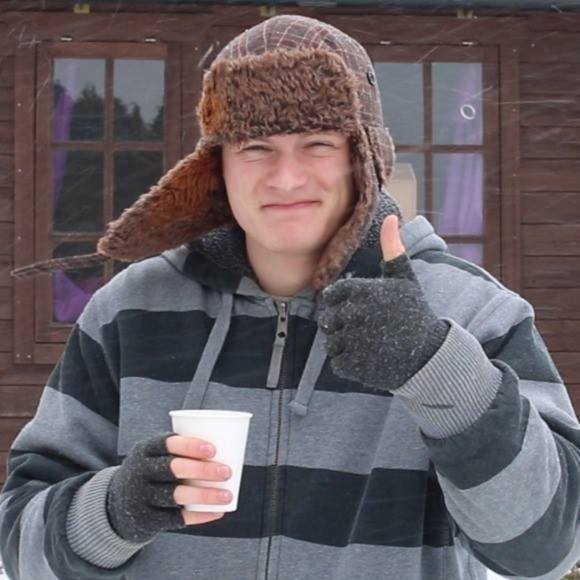