How Power Electronics Work: A Detailed Guide
23-04-2024 | By Robin Mitchell
Introduction
Power electronics play a crucial role in modern electrical engineering, essential for efficiently converting, controlling, and managing electrical power within circuits. These components are designed to handle high voltages, currents, powers, and temperatures, making them vital for various industries. Within power electronics, components are broadly categorized into passive components, such as power resistors, diodes, inductors, and capacitors, and active components, including MOSFETs, IGBTs, and BJTs. With advancements in technology and materials, Silicon Carbide (SiC) and Gallium Nitride (GaN) materials are expected to dominate the future landscape of power electronics. Before exploring these current technologies and their applications, consider taking a moment to understand the history of power electronics, which highlights significant advancements that pave the way for today's innovations. For those newer to the subject, you might also find it useful to start with a primer on what power electronics are to build a solid foundation.Â
Table of contents:
- Introduction
- Components of Power Electronics
- Why Power Electronics Are Needed
- Rectification - From AC to DC
- Inversion - Converting DC to AC
- AC Conversion
- DC Converters - Regulators
- DC Converters - Buck & Boost
- Mechanical Control
- Electronic Control
- Filtering
- Protection
Components of Power Electronics
Passive Components
Passive components, like power resistors, are critical in power electronics as they provide resistance to current flow in a circuit. These resistors can handle high power levels and efficiently dissipate heat to prevent overheating. Proper selection of power resistors is crucial in high-power applications like motor drives or power supplies to ensure system reliability and performance.
Diodes are another essential passive component in power electronics, allowing current flow in one direction while blocking it in the opposite direction. Diodes are ideal for rectification in AC to DC conversion, voltage regulation, and protection against voltage spikes. In high-power applications such as electric vehicles or renewable energy systems, diodes with high voltage and current ratings ensure efficient power conversion and system reliability.
Inductors store energy in the form of a magnetic field when current flows through them. In power electronics, inductors are used in DC-DC converters, filters, and energy storage applications. Design considerations for inductors in high-power systems include core material selection, wire gauge, and thermal management to minimize power losses and maximize efficiency.
Capacitors store and release electrical energy in the form of an electric field. In power electronics, capacitors are used for smoothing voltage ripples, filtering noise, and providing energy storage. High-power capacitors are designed to withstand high voltages and currents while maintaining stable capacitance values over a wide temperature range for reliable operation in demanding environments.
Active Components
Moving on to active components, Metal-Oxide-Semiconductor Field-Effect Transistors (MOSFETs) control current flow based on the voltage applied to the gate terminal. MOSFETs are widely used in power electronics for switching applications due to their high efficiency, fast switching speeds, and low conduction losses, crucial for precise power flow control and energy efficiency in high-power systems.
Insulated Gate Bipolar Transistors (IGBTs) combine the high input impedance of MOSFETs with the low on-state voltage drop of bipolar transistors, making them suitable for high-power switching applications. IGBTs play a key role in controlling power flow and regulating voltage levels efficiently in motor drives, renewable energy systems, and industrial power supplies.
Bipolar Junction Transistors (BJTs) consist of three doped regions and are used for amplification and switching of electrical signals. While not as commonly used in power electronics as MOSFETs and IGBTs, BJTs find applications in audio amplifiers, RF circuits, and low-power control systems due to their high current gain and switching speed advantages.
Looking towards the future, Silicon Carbide (SiC) and Gallium Nitride (GaN) materials are expected to bring significant improvements to power electronics. SiC offers higher breakdown voltage, lower on-state resistance, and better thermal conductivity, ideal for high-power and high-temperature applications. GaN provides higher electron mobility, lower switching losses, and faster switching speeds, enabling compact and efficient power electronic systems in aerospace, automotive, and renewable energy sectors, driving innovation towards more energy-efficient systems with reduced size, weight, and cost.
Why Power Electronics Are Needed
Applications in Daily Life
In order to grasp the crucial role of power electronics within the electronics field, it's helpful to look at a common household scenario. Homes typically receive electricity as AC at high voltages, ranging from 110V to 230V. While this high-voltage AC power is effective for operating appliances such as refrigerators and cookers, it's not suitable for sensitive low-voltage electronic devices, including computers and laptops, which require DC power to function safely and efficiently. This necessitates the use of a converter to transition the incoming AC power to DC power.
Furthermore, devices like laptops and smartphones that incorporate batteries for mobility, rely on power electronics for the regulation of charging voltage and current to maximize battery life and ensure safety. The significance of power electronics extends well beyond personal devices at home; they are integral in sectors including electric vehicles (EVs), power stations, and industrial settings. Essentially, any scenario that involves electricity conversion, from adjusting voltage levels to converting between AC and DC or managing power flow, depends on power electronics. Their extensive application highlights the indispensable role power electronics serve in contemporary electronic systems.
Industrial and Commercial Applications
For electric vehicles, power electronics are critical in directing power flow among the battery, motor, and other components, adjusting the motor's speed and torque by modulating power from the battery. They also enable regenerative braking, converting kinetic energy back into electrical energy for battery storage.
Power stations rely on power electronics for the efficient generation, transmission, and distribution of power. Devices such as transformers, inverters, and rectifiers are employed to modify electricity to appropriate voltage levels for transmission and distribution. Furthermore, in renewable energy systems like solar and wind power, power electronics convert the variable DC output into AC power compatible with the grid.
In industrial environments, power electronics facilitate motor control, heating systems, lighting control, and various automation processes, allowing for energy savings and enhanced efficiency. They are also crucial in improving power quality, addressing issues like harmonics and voltage fluctuations to ensure the stable operation of industrial equipment.
Additionally, power electronics are vital in commercial settings, including datacenters and healthcare institutions, by providing uninterruptible power supply (UPS) systems. These systems ensure continuous power during outages, protecting critical equipment and data. UPS systems utilize power electronics to seamlessly switch between AC mains power and battery backup, offering a dependable power source in emergencies.
The pervasive application of power electronics across various sectors from residential to industrial, highlights their fundamental importance in facilitating efficient power conversion, control, and management. Power electronics are thus a foundational element in ensuring the safe and reliable functioning of electronic devices and power systems, underscoring their indispensability in our interconnected world.
Rectification - From AC to DC
Basic Rectification Processes
Rectification is a crucial process in electronics that involves converting alternating current (AC) into direct current (DC). This conversion is essential for many electronic devices to function properly, as most devices operate on DC power. The process of rectification is typically achieved using a rectifier, which is a component that allows current to flow in only one direction.
One of the simplest forms of rectification is the half-wave rectifier, which utilizes a single diode. The diode acts as a one-way valve, allowing current to flow during the positive half-cycle of the AC signal while blocking it during the negative half-cycle. While effective, half-wave rectifiers have limitations in terms of efficiency and smoothness of the output waveform.
To overcome the limitations of half-wave rectifiers, full-wave rectifiers are often used. A full-wave rectifier configuration employs four diodes arranged in a specific manner to ensure that current flows in the same direction during both the positive and negative half-cycles of the AC signal. This results in a more constant DC output with less ripple compared to half-wave rectifiers.
In practice, rectifier packages are available that integrate all the necessary diodes and circuitry into a single component. These integrated rectifiers simplify circuit design and assembly, making them ideal for applications where space and complexity need to be minimized.
The use of rectifiers is widespread in devices that need to draw power from AC sources, such as computers, smartphones, and various IoT devices connected to mains power. Without rectification, these devices would not be able to operate efficiently or safely.
Advancements and Efficiency
Efficiency is a significant concern when it comes to rectifiers. Diodes, which are the key components in rectifiers, have a forward voltage drop that results in energy being dissipated as heat. This not only reduces the overall efficiency of the rectifier but can also lead to heating issues, especially in high-power applications.
To address the efficiency and heat dissipation challenges, Schottky diodes are often used in rectifier circuits. Schottky diodes are constructed using a piece of N-type semiconductor material and a metal conductor, which gives them a very low forward voltage drop (typically between 0.1V to 0.3V). This low forward voltage drop minimizes energy loss and heat generation in the rectifier.
However, using Schottky diodes comes with trade-offs. While they offer high efficiency, they have limitations in terms of maximum reverse voltage and high reverse currents. This means that Schottky diodes are best suited for applications where efficiency is critical, and the maximum operating voltage requirements are within their specified limits.
Rectification plays a vital role in converting AC power to DC power for various electronic devices. By understanding the different rectifier configurations and the importance of efficiency in rectifier design, engineers can choose the right components to ensure optimal performance and reliability in their electronic systems.
Inversion - Converting DC to AC
Basic Inversion Techniques
Inversion is the process of changing direct current (DC) to alternating current (AC), which serves as the opposite of rectification, where the conversion happens in the reverse direction. This shift from DC to AC involves the use of different components and technologies to ensure a steady and dependable AC power output.
At its most basic form, inverters can use a switched DC current to produce an output waveform similar to a square wave, known as a modified sine wave generator. While this waveform may be suitable for some AC devices, it can create difficulties for more delicate electronics that depend on the consistent and smooth AC waveforms typically provided by mains voltages. The irregularities in the waveform produced by modified sine wave inverters can cause operational issues and decreased performance in devices that need a stable AC input.
To meet the needs of sensitive electronic equipment, more advanced inverters are designed to replicate a sine wave output. By imitating the sinusoidal waveform of standard AC power, these sophisticated inverters allow delicate devices to operate seamlessly on such power supplies. This simulation of a sine wave is often accomplished through an array of Metal-Oxide-Semiconductor Field-Effect Transistors (MOSFETs), with each component managing a specific part of the waveform to ensure a coherent output.
Inverters are essential in renewable energy generation systems, such as solar panels and wind turbines, where the primary output is DC current. By converting the produced DC power into AC, inverters make it possible to integrate renewable energy sources into existing AC grids, making the distribution and utilization of clean energy efficient.
Enhancing Inverter Efficiency
Despite their significance in energy conversion, achieving high energy efficiency with inverters can be a demanding task. The use of switched devices in inverters, which function in on-off modes, can lead to notable energy losses. On the other hand, operating devices in linear modes would be more energy-efficient but may not always be possible due to technical limitations.
Top-quality sine wave inverters have the potential to achieve efficiency levels of up to 95%, ensuring minimal energy wastage during the conversion process. In contrast, modified sine wave inverters typically struggle to surpass 80% efficiency, emphasizing the importance of selecting the suitable inverter technology based on the specific needs of the application.
Additionally, many inverters are used alongside low-voltage systems, like batteries ranging from 12V to 36V. In such setups, the significant current flow needed to power devices can result in substantial heating of cables, further reducing the overall efficiency of the system. The resistance of the cables to the current passing through them leads to energy loss in the form of heat, highlighting the necessity for effective cable management and heat dissipation strategies in inverter installations to enhance performance and reliability.
AC Conversion
The modification of voltage in the field of electrical engineering entails adjusting one voltage source to align with the intended application. Unlike rectifiers, which change AC to DC or vice versa, converters typically retain the type of current being altered, thereby preserving the AC or DC traits of the initial signal. This distinction is crucial as it dictates the components and circuits utilized in the alteration process.
Role of Transformers
In stages of AC voltage alteration, transformers play a crucial role. These devices possess the ability to either increase or decrease the voltage level of the input signal. A transformer comprises two coils of wire, referred to as the primary and secondary coils, wound around a shared magnetic core. When an alternating current passes through the primary coil, it induces a changing magnetic field due to electromagnetic induction. This changing magnetic field, in turn, generates a current in the secondary coil.
One of the fundamental principles of transformers is that the power input always equals the power output, accounting for some losses due to inefficiencies in the alteration process. This implies that if the voltage is increased by a transformer, the output current will be proportionally reduced to maintain power balance. Conversely, if the voltage is decreased, the output current will increase proportionally to uphold power equilibrium.
The efficiency of transformers is a crucial consideration in AC voltage alteration. While ideally, the input power should match the output power, losses occur in practical scenarios due to factors like resistance in the coils, eddy currents, and hysteresis losses in the core material. These losses lead to a decrease in the efficiency of the transformer and can result in heat generation, impacting the overall performance of the alteration system.
Furthermore, transformers are engineered to function within specific voltage and current limits to prevent overheating and damage. Overloading a transformer beyond its rated capacity can lead to excessive current flow, resulting in overheating of the coils and insulation, potentially causing the transformer to fail. Therefore, proper sizing and selection of transformers based on the input voltage, output requirements, and load characteristics are crucial for effective and reliable AC voltage alteration.
In practical applications, transformers are utilized in various electrical devices and systems to adjust voltage levels for the transmission, distribution, and utilization of electrical power. For example, in power distribution networks, step-up transformers are utilized to raise the voltage for efficient long-distance transmission, while step-down transformers are employed to lower the voltage for safe and suitable use in homes and industries.
The design and integration of transformers in AC voltage alteration systems necessitate careful consideration of factors such as insulation, core material, winding configurations, and cooling mechanisms to ensure optimal performance and longevity. Progress in transformer technology, such as the use of high-efficiency materials, enhanced insulation techniques, and smart monitoring systems, have contributed to improving the reliability and efficiency of AC voltage alteration processes in modern electrical systems. By grasping the principles of transformer operation and their role in AC voltage alteration, engineers can develop creative solutions for various applications in power electronics and electrical engineering.
DC Converters - Regulators
DC converters, as the name suggests, are electronic devices created to adjust one direct current (DC) voltage to another. This alteration process is crucial in various electronic applications where varying voltage levels are necessary for different components to function effectively. Similar to alternating current (AC) converters, DC to DC converters can either increase or decrease a DC voltage level, depending on the specific needs of the circuit or system.
Linear vs. Switching Regulators
One of the most basic types of DC converters is the linear regulator. Linear regulators are widely used in electronics for their simplicity and efficiency in reducing a higher input voltage to a lower output voltage. These regulators function by releasing the excess voltage as heat, thereby decreasing the output voltage to the desired level. While linear regulators are recognized for their low output noise features, especially when compared to other types of regulators, they have a notable downside in terms of energy efficiency.
The inefficiency of linear regulators arises from their operational principle. Since linear regulators release excess voltage as heat to achieve the desired output voltage, they are inherently inefficient in terms of energy. For example, consider the well-known AMS1117 linear regulator. If the regulator needs to provide a 1A current output with a voltage difference of 5V between its input and output, it will waste at least 5 watts of power in the form of heat. This energy wastage can be a crucial factor in battery-operated devices or in applications where energy efficiency is a primary concern.
In practical terms, the inefficiency of linear regulators can result in several problems in electronic systems. The wasted energy is not just a financial concern due to increased power consumption but also contributes to thermal management challenges. The heat produced by the linear regulator must be dissipated effectively to prevent overheating, which can impact the overall reliability and lifespan of the electronic components.
To tackle the energy efficiency challenges presented by linear regulators, engineers frequently explore alternative solutions such as switching regulators. Switching regulators function on a different principle than linear regulators, where they alternate the input voltage on and off rapidly to regulate the output voltage efficiently. This switching action enables switching regulators to achieve higher energy efficiency compared to linear regulators, making them a favoured choice in many contemporary electronic designs.
Additionally, in addition to energy efficiency considerations, the selection between linear and switching regulators also involves factors such as cost, size, and electromagnetic interference. While linear regulators are generally simpler and more economical, switching regulators offer superior efficiency and are more suitable for applications where energy conservation is crucial.
In practical terms, while linear regulators serve as a fundamental building block in many electronic circuits due to their simplicity and low noise characteristics, their inefficiency in energy conversion poses challenges in modern electronic designs. Engineers must carefully assess the requirements of their applications and consider factors such as power consumption, heat dissipation, and overall system efficiency when choosing the appropriate DC converter or regulator for a given project. By evaluating the advantages and limitations of different types of regulators, engineers can optimize the performance and energy efficiency of their electronic systems.
DC Converters - Buck & Boost
Buck Converters
Instead of relying solely on traditional linear regulators to decrease voltage, engineers often turn to more efficient solutions like the buck converter. Unlike linear regulators that dissipate excess power as heat, buck converters switch the power source on and off rapidly, utilizing inductors and capacitors to smooth out the switched power and provide a lower output voltage. The efficiency of buck converters can reach up to an impressive 99%, making them a popular choice in various electronic applications.
However, one significant drawback of buck converters is their tendency to generate high levels of electromagnetic interference, which can interfere with the operation of sensitive logic components like microcontrollers. This noise can lead to malfunctions or even complete failure of the electronic system. To mitigate this issue, engineers often combine a buck converter with a linear regulator. The buck converter first decreases the voltage efficiently, and then the linear regulator further refines the output, providing a smooth and reliable power supply to the sensitive components.
Boost Converters and Noise Management
Conversely, when a voltage boost is required, engineers turn to boost converters. Boost converters operate by using capacitive coupling and high-frequency switched devices to increase the input voltage to a higher output voltage level. While boost converters are effective in increasing voltages, they also suffer from the same noise-related challenges as buck converters. The high-frequency switching action can introduce significant noise on the power rails, which can be detrimental to the proper functioning of the electronic system.
To address the noise issues associated with boost converters, engineers must implement various filtering techniques. This includes integrating multiple filter components into the design, ensuring a robust ground plane for proper grounding, and in some cases, incorporating additional filter stages to suppress the noise effectively. By carefully designing the layout and incorporating the necessary filtering components, engineers can minimize the noise generated by boost converters and ensure the overall stability and reliability of the electronic system.
Mechanical Control
Control devices play a pivotal role in managing electricity flow across various systems, with mechanical switches standing as a foundational component in this domain. These switches, ranging from the small buttons on a smartphone to the large levers used in grid distribution sites, are crucial for their reliability and ease of use, particularly in scenarios that demand direct human interaction or when managing high electrical currents more effectively through mechanical means rather than electronic solutions.
Relays and Switches
For high voltage applications, such as substations that deal with hundreds of thousands of volts, mechanical switches are indispensable. Large levers, operated manually, are employed to connect or disconnect circuits—a task that electronic switches like transistors are ill-equipped to handle due to the extreme voltage and currents. Additionally, relays, which operate on electromagnetism to direct the flow of electricity, are invaluable for intermittently managing high currents. Their applications are widespread, found in telephone exchanges, power systems, and the pioneering computers of the past.
Relays, having been utilized for an extended period, have proven their adaptability and dependability across various settings. Capable of managing significant power loads, they come in numerous forms and sizes, catering to a broad spectrum of applications, from electric vehicles and DC motor controllers to lighting systems. However, relays are not without their flaws. One of the main issues is contact welding, where the relay's mechanical contacts fuse together, rendering the relay incapable of opening or closing as required.
This phenomenon of contact welding presents a formidable challenge. Once the contacts are welded together, separating them without causing further damage to the relay is often difficult. This can be particularly problematic in essential systems, where a relay's failure to open could have hazardous consequences. Therefore, despite their extensive application and overall reliability, the issue of contact welding underscores a critical vulnerability in mechanical control devices, marking an area in need of attention to ensure the safety and efficiency of electrical systems.
Electronic Control
Transistors in Power Control
Active electronic components play a crucial role in power management within electronic systems, with transistors being key among these components. Transistors, including types such as MOSFETs, FETs, BJTs, and IGBTs, serve as active switches to regulate current flow, offering a range of benefits and drawbacks that merit careful consideration.
One of the primary advantages of employing transistors in power control is their solid-state construction. This attribute means transistors lack moving parts, which significantly reduces wear and tear compared to mechanical switching devices. As such, transistors are more reliable, especially in applications where minimizing the risk of welding or contact failures is critical.
Additionally, the compact and lightweight nature of transistors allows for their integration into small electronic devices, enhancing portability and efficiency. Their rapid switching capability is particularly beneficial in digital switching applications, such as Switched-Mode Power Supplies (SMPS) and converters, where precise and quick power control is essential.
However, transistors are not without their challenges. They are generally more suited to low-power applications due to typically lower voltage ratings, which makes handling high-power applications above 1500V difficult. The complexity of driving transistors efficiently can also introduce power control inefficiencies, impacting system performance.
Furthermore, transistors can be susceptible to dangerous conditions like thermal runaway and avalanches if operating conditions exceed safe limits, threatening system stability and reliability.
Another important factor to consider is that transistors are primarily designed for DC current control and do not fare well with AC systems. This limitation narrows their use in certain power control scenarios, necessitating alternative approaches for AC power regulation.
Therefore, while transistors bring significant advantages to power control applications, such as enhanced reliability, compactness, and fast switching, acknowledging and mitigating their limitations is essential. By evaluating the specific needs of an electronic system and weighing the advantages and disadvantages of transistors, engineers can make informed decisions to optimize power management and ensure the efficacy and safety of the electronic control system.
Filtering
Importance of Filtering in Circuits
Managing and converting power is a crucial part of electronic design, but the significance of filtering in guaranteeing the stability and dependability of a system cannot be emphasized enough. Filtering plays a vital role in refining the power from a source by getting rid of noise, unexpected transients, and smoothing out ripples that could otherwise disturb the operation of electronic devices.
In the field of filtering, components are mainly passive in nature and include inductors, capacitors, and resistors. These components collaborate to enhance the quality of the power being delivered to the circuitry.
Inductors, for example, are typically used to counteract large transients in current and voltage. Positioned in series with a voltage source, inductors act as a barrier against sudden spikes in current or voltage that may arise. For instance, in the scenario of a lightning strike hitting a nearby electrical grid, an inductor in series can help alleviate the surge of current resulting from the strike, protecting the connected devices from potential damage.
On the other hand, capacitors are essential in eliminating noise by smoothing out variations in voltage. This process, known as decoupling, involves allowing the capacitor to charge during normal operation and discharge rapidly in response to sudden voltage drops from the source. Decoupling capacitors also function as bypass circuits for high-frequency signals, guaranteeing that these signals do not disrupt the normal functioning of the circuit.
For instance, in the situation of a switch-mode power supply (SMPS) that experiences high-frequency switching, it can introduce disturbances, or "fuzz," on the powerline. If these disturbances reach a sensitive component like a microcontroller, they can lead to erratic behavior. To prevent this, a small-valued capacitor, such as a 1nF capacitor, placed in parallel can effectively create a path for the high-frequency signals to be directed to the ground, thereby isolating them from affecting the overall circuit operation. By strategically integrating filtering components like capacitors and inductors, designers can ensure that the power supplied to electronic systems is stable, free from interference, and conducive to optimal performance.
Protection
Protective Devices in Power Electronics
Securing electronic systems requires a deep understanding and careful consideration of protection in power electronics. Protective devices are crucial in ensuring that all connected devices are shielded from potential damage or malfunction in case of unexpected events like a sudden surge of current. Among the various protective mechanisms, the fuse is commonly used in safeguarding electronic systems.
Fuses are essential components in protection as they break the circuit when the current exceeds a set threshold. They come in various forms to suit different needs and situations. Wire fuses break physically when the current goes beyond the rated value, thus interrupting the circuit. On the other hand, polyfuses reset themselves after a cooling period following a tripping event. Additionally, mechanical trip switches are another type of fuse that requires manual resetting after activation.
Protective components like Zener diodes are valuable assets in shielding electronic circuits from potential harm. When used in reverse bias mode, Zener diodes clamp the input voltage, preventing voltage spikes from reaching sensitive components. For example, a Zener diode rated at 5.1V diverts surplus current if the output voltage exceeds the 5.1V threshold. It is crucial to include a series current limiting resistor with Zener diodes to prevent failure due to excessive current flow.
The use of Zener diodes as protective elements is highly effective in protecting circuits against overvoltages that could damage crucial components within the system. By strategically integrating Zener diodes into the circuit design, engineers can enhance the resilience and longevity of electronic systems, fortifying them against unforeseen electrical anomalies and fluctuations that could compromise their functionality.
Therefore, incorporating protective devices like fuses and Zener diodes is a proactive measure in strengthening electronic systems against potential hazards and disturbances. By implementing these safeguards, engineers can enhance the resilience and reliability of their designs, ensuring the longevity and optimal performance of the systems even in the face of unpredictable events or electrical irregularities.
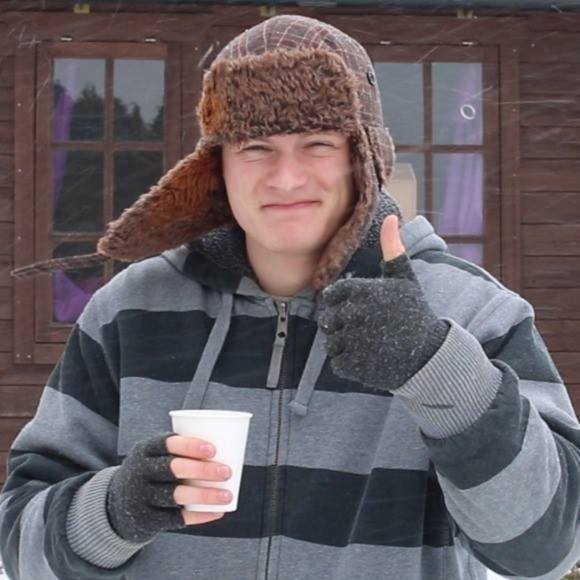